Absorbance Spectroscopy
In absorbance spectroscopy (also known as absorption spectroscopy), a spectrometer is used to directly measure how much light is absorbed by a sample as a function of wavelength.
Quantifying the absorbance of light by an atom or molecule can provide important information about the molecules electronic structure. Depending on the sample, absorbance measurements can also give you key insights into other material properties, such as sample concentration, phase changes, or composition changes.
Spectrometer and Accessories

- Low Price Optical Spectrometer
- Light Sources
- And More
Complete Kits for £2,400
Absorbance Theory
Band theory describes how electrons are organised within a solid. This can be useful when discussing different properties of a solid (conductors, semiconductors, or metals).
Within an atom, electrons can exist in regions around the nucleus known as orbitals. Depending on the electronic structure of an atom, these orbitals (or energy levels) can be filled, partially filled, or empty. The wavelength of light that can be absorbed by a material is dependent on these different energy levels and the electronic distribution within this material.
Absorption occurs when the the energy of the incoming photon is equal or larger than energy difference (ΔE) between the highest occupied molecular orbit (HOMO) and the lowest unoccupied molecular orbit (LUMO).
If the photon energy is high enough, it can be absorbed by an electron in the HOMO and will be excited into a higher energy state.
The wavelengths of the absorbed light correspond to energy through the equation.
When multiple atoms come together to form molecules, energy levels become more complex. For example, the types of electronic transitions that are allowed will often vary with different material properties. Measuring the wavelengths at which absorbance occurs will therefore tell you a lot about the electronic properties of a material, molecule or thin film.
Absorbance Measurement
To measure absorbance requires a broadband light source and a spectrometer with appropriate spectral range. Absorbance values are closely related to transmission measurements, as in both cases you actually measure the transmitted light.
As light is propagated through a sample and absorbed, its intensity will fall exponentially with distance. You can calculate absorbance by the comparing the reference light spectrum, I0, tothe detected light that has passed through the sample, I, through this equation.
Defining Absorbance
Absorbance is a relative measurement so is therefore unitless. This absorbance, A, can be defined in a number of different ways for different samples.
Molar Attenuation Coefficient
When you measure the absorbance spectrum of a solution, it is important to at which wavelengths maximum absorbance occurs. Identifying these wavelengths can can help you determine certain molecular properties of your sample. It is also important to note the strength of absorbance at these wavelengths.
By looking at these peaks, you can establish the molar attenuation coefficient, ε. This has also been referred to historically as the molar extinction coefficient and is also known as the molar absorptivity coefficient. This will tell you about the kinds of electronic transitions that are occurring within your sample associated. For allowed transitions, ε > 1000 whereas for forbidden transitions, ε < 100.
The molar attenuation coefficient, ε, is featured in Beer Lambert's Law:
Where A is absorbance, c is the molar concentration of the molecule in solution, and l is the path length through the sample (often the width of the cuvette, or the total film). You can use this calculation to measure the concentration of a molecule in a thin film. The graphs below show the variation in absorbance intensity with concentration. This is a linear relationship.
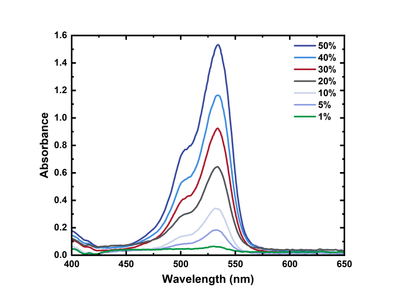

Molar attenuation coefficient has property has dimensions of
Therefore, the SI units of the molar absorptivity coefficient is m2M-1 or cm2M-1.
Absorption Coefficient
For bulk solids, you can define absorbance in terms of an absorption coefficient, α(λ). This is a property of a solid material and is wavelength dependent. The absorption coefficient, α(λ) relates to absorbance measurements through the following equation:
Here, d is the distance light has travelled into (or through) the bulk solid.
The SI units of the absorption coefficient of a bulk solid are m-1 or cm-1.
Absorbance Units
Property | Units | Sample Type |
---|---|---|
Absorbance | Unitless | General |
Absorption Coefficient | m-1 | Bulk Solid |
Molar Absorptivity Coefficient | m2M-1 | Molecule in solution |
Absorbance Uses
You can measure UV-Vis absorbance to investigate the suitability of a material for a specific purpose or to determine its material properties. For example:
- Organic dyes require high absorbance over a small wavelength range so that only light of a matching colour is emitted from the dye, e.g. for a yellow dye, blue light (435 - 480 nm) must be absorbed.
- Materials that convert visible light into different forms of energy (such as solar cells, photosensors) will require high absorption, ideally across the visible region. These materials frequently have desirable band gaps, which can be determined using UV-Vis spectroscopy.
- Transparent materials need to absorb as little visible light as possible. You can confirm this using UV-Vis spectroscopy.
- In organic compounds, UV-vis spectroscopy can help illuminate the amount of conjugated pi bonds in a molecule.
Spectrometer and Accessories

- Low Price Optical Spectrometer
- Light Sources
- And More
Complete Kits for £2,400
References and Resources
- Rocha, F. S., Gomes, A. J., Lunardi, C. N., Kaliaguine, S., & Patience, G. S. (2018). Experimental methods in chemical engineering: Ultraviolet visible spectroscopy-UV-vis. The Canadian Journal of Chemical Engineering, 96(12), 2512–2517. DOI:10.1002/cjce.23344
- Hestand, N. J., & Spano, F. C. (2018). Expanded theory of H- and J-molecular aggregates: The effects of vibronic coupling and intermolecular charge transfer. Chemical Reviews, 118(15), 7069–7163. DOI: 10.1021/acs.chemrev.7b00581
- Makuła, P., Pacia, M., & Macyk, W. (2018). How to correctly determine the band gap energy of modified semiconductor photocatalysts based on UV–vis spectra. The Journal of Physical Chemistry Letters, 9(23), 6814–6817. DOI: 10.1021/acs.jpclett.8b02892
- Chen, J., Zhou, S., Jin, S., Li, H., & Zhai, T. (2016). Crystal organometal halide perovskites with promising optoelectronic applications. Journal of Materials Chemistry C, 4(1), 11–27. DOI: 10.1039/c5tc03417e